Main Content
Research profile
Chemistry at surfaces and interfaces for energy harvesting, storage, and transformation:
Surface analysis, Photoelectron spectroscopy, Scanning probe microscopy, X-ray absorption spectroscopies, Molecular and ion beam techniques
We study chemical reactions at complex surfaces and interfaces with spectroscopic, microscopic, and molecular beam / ion beam techniques. The experiments are performed on well-defined surfaces of solids, thin films, or liquids in ultrahigh vacuum (UHV). Our research covers fundamental and applied aspects with focus on energy harvesting, storage, and transformation.
Current projects
Current research areas include (a) Elementary steps of surface reactions related to energy harvesting, storage, and transformation, (b) New energy materials through on-surface synthesis, (c) Internal metal-organic interfaces for organic electronics and renewable energy applications, (d) Model catalysis, (e) Functional nanomaterials with novel electronic, chemical and catalytic properties, and (f) Development of novel techniques for surface and interface science.
Methods
We use a wide range of spectroscopic, microscopic, diffraction, and molecular/ion beam techniques: Photoelectron Spectroscopy (XPS, UPS, HAXPES), X-Ray Absorption Spectroscopy (NEXAFS, XANES), Scanning Tunneling Microscopy (STM), Low-Energy Electron Diffraction (LEED), Nanojoule Adsorption Calorimetry (NAC), Temperature Programmed Desorption (TPD), Molecular Beam and Ion Beam Deposition Techniques. Many measurements are performed with synchrotron radiation. For the in-depth interpretation of these results, we also perform density-functional theory (DFT) calculations.
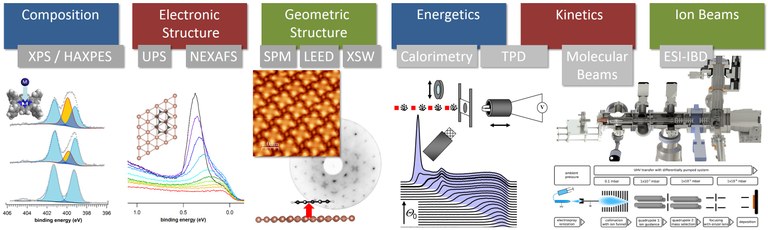
Figure. Experimental methods. (Click to enlarge)
Research Examples
1. Carbon-Based Functional Nanomaterials by On-Surface Synthesis
Graphene derivatives such as graphene nanoribbons and cyclic nanographene have enormous potential as functional materials in (opto)electronic devices and other energy-related applications. The band gap and other important properties depend critically on the structure and especially the edge terminate of the graphene nanostructures. Therefore, only an atomically precise bottom-up synthesis leads to products with reproducible properties. We use surfaces as catalysts and templates for the fabrication of carbon nanoribbons and related produces such as cyclic nanographenes. In addition, we study related molecular, supramolecular, organometallic and covalent polymer nanostructures. A particularly beautiful example for supramolecular structures based on halogen compounds is given by the Sierpinski triangle fractals.
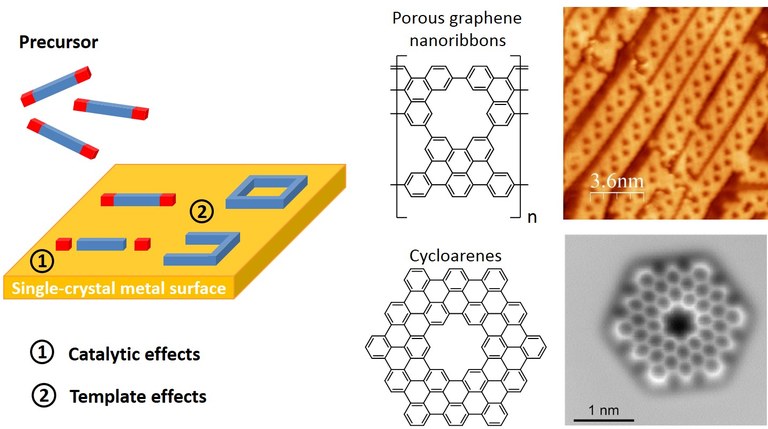
Figure. Principle of on-surface synthesis and examples for applications. (Click to enlarge)
Further reading:
Q.T. Fan et al., J. Am. Chem. Soc. 142 (2020) 894
Q.T. Fan et al., J. Am. Chem. Soc. 141 (2019) 17713, highlighted in Nature
Q.T. Fan et al., Nature Comm. 10 (2019) 5049
Q.T. Fan et al., J. Am. Chem. Soc. 140 (2018) 7526
M. Chen et al., ACS Nano 11 (2017) 134
Q.T. Fan et al., ACS Nano 10 (2016) 3747
J. Shang et al., Nature Chem. 7 (2015) 389
Q.T. Fan et al., ACS Nano 8 (2014) 709
Q.T. Fan et al., Angew. Chem. Int. Ed. 52 (2013) 4668
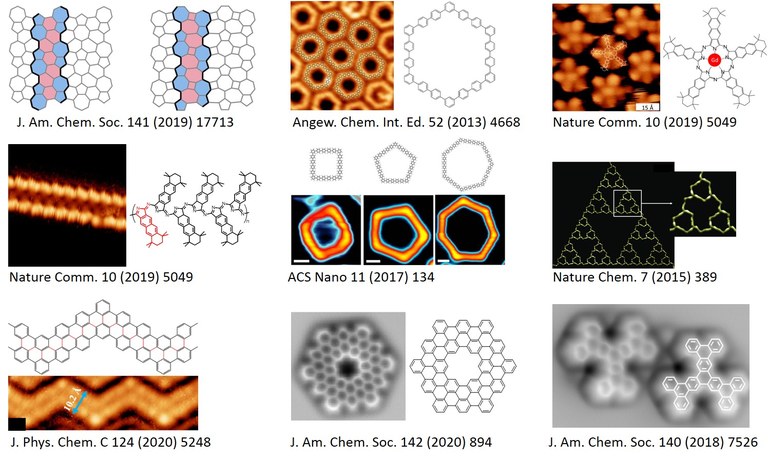
Figure. On-surface synthesis of carbon-based nanostructures, recent examples. (Click to enlarge)
2. Internal (Buried) Interfaces: Electrodes, Metal/Organic Interfaces, Semiconductor Interfaces
Interfaces between metals and molecular or polymeric organic semiconductors play an important role in organic photovoltaics and other electronics applications. The alignment of energy levels and the overlap of wavefunctions are decisive for the efficient transfer of charge carriers at the interface. We study internal metal/organic interfaces and focus particularly on chemical reactions and diffusion processes in the interface region. The transition from an inert, smooth interface to the formation of diffusion and reaction zones (interphases) depends on the properties of the pure phases as well as on details of the preparation process. Electronic, chemical, energetic and kinetic effects of the interface formation are studied with a wide range of spectroscopic and other techniques. Related topics of our research on internal (buried) interfaces include inorganic semiconductor interfaces and solid state electrodes. Studies at buried interfaces are challenging, because surface-sensitive techniques cannot be used and the (usually weak) signal from the interface region must be separated from the (usually dominating) signals from the bulk phases. Suitable methods include Hard X-ray Photoelectron Spectroscopy (HAXPES), X-ray Absorption Spectroscopy (XAS/NEXAFS), molecular beam techniques, and nanojoule calorimetry. This project is supported by the Collaborative Research Center SFB 1083.
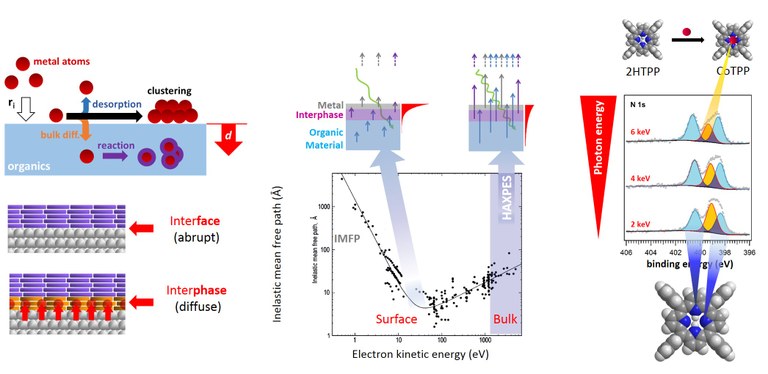
Figure. Left, dynamics of interface formation resulting in abrupt or diffuse interfaces. Center, chemical depth profiling with Hard X-ray Photoelectron Spectroscopy (HAXPES). Right, HAXPES study of a reactive, diffuse metal/organic interface. (Click to enlarge)
Further reading:
M. Schmid et al., J. Phys. Condens. Matter 31 (2019) 094002
M. Schöniger et al., Chem. Comm. 55 (2019) 13665
J.M. Gottfried, U. Höfer, J. Phys. Condens. Matter 31 (2019) 500301
M. Chen et al., Phys. Chem. Chem. Phys. 18 (2016) 30643
M. Sachs et al., Phys. Chem. Chem. Phys. 17 (2015) 31790
3. Molecular Topology and Metal-Organic Interfaces
How does the interaction of aromatic molecules with metals depends on the topology of their π-electron systems? Interfaces between π-conjugated organic semiconductors and metal electrodes play a prominent role in organic (opto)electronic devices and critically influence their performance. Rational strategies for the improvement of these devices therefore require a detailed understanding of the chemical, electronic and geometric interface structure. In the example below, we use the isomers azulene, which has a nonalternant π-system with a 5-7 ring structure, and naphthalene, which has an alternant π-system with a 6-6 ring structure. By measuring adsorption heights (with NIXSW) and adsorption energies, we find that azulene interacts much more strongly with a metal surface. Related differences were observed in the electronic structure of the two interfaces. Similar trends were found for other pairs of alternant/non-alternant isomers. Our results indicate that the electronic properties of metal/organic interfaces can be adjusted through modifications of the π-topology of the organic semiconductor.
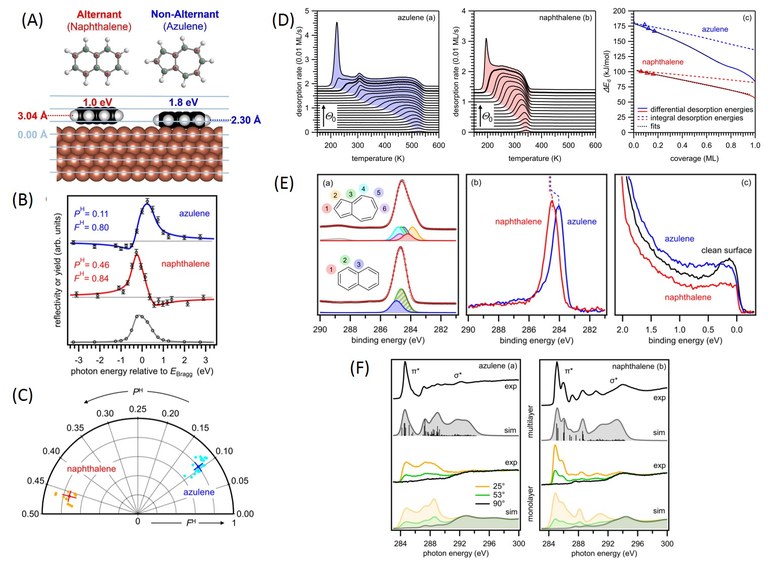
Figure. (A) Alternant (naphthalene) and non-alternant (azulene) π-electron systems on a copper (111) surface with experimental adsorption heights from NIXSW measurements (B,C) and adsorption energies from TPD (D). (E) Photoelectron spectroscopy (XPS, UPS) and (F) NEXAFS reveal details of the surface chemical bond, which depends strongly on the topology of the conjugated π-electron systems. (Click to enlarge)
Further reading:
S.R. Kachel et al., J. Phys. Chem. C 124 (2020) 8257
B.P. Klein et al., Chem. Mater. 32 (2020) 1041
B.P. Klein et al., J. Phys. Chem. C 123 (2019) 29219
B.P. Klein et al., Phys. Rev. X 9 (2019) 011030
4. Surface Functionalization with Redox-Active Metal Complexes as Potential Electrocatalysts
This project deals with the functionalization of solid surfaces with nanostructured layers of adsorbed, reactive metal complexes as potential (electro)catalysts. As a suitable class of model systems, we study the interaction of metalloporphyrins, metallocorroles and related planar metal complexes with metals, oxides, and nanoporous materials. The metal center in the complex as a carrier of the functionality is strongly influenced by adsorbate-substrate interactions. We focus especially on changes of the electronic state and related modification of reactivity. In addition, we study the binding of small reactive molecules such as NO, NH3, CO und O2 on the metal center, which is an important reaction for single-site (electro)catalysis and sensor applications. The bond to this new ligand competes with the bond to the underlying surface. This surface trans effect can be used to control the properties of the metal center. The complex bonding situation can be clarified by studies with photoelectron spectroscopy (XPS/UPS) and DFT calculations, combined with synchrotron-based X-ray standing waves (XSW) measurements to determine the adsorbate-substrate bond distances.
A versatile on-surface reaction of the ligand molecules is the in-situ metalation, which leads to metal(2+) complexes with porphyrins and phthalocyanines, but to metal(3+) complexes with corroles. Mechanistic details of these reactions are clarified by spectroscopic and microscopic measurements, in combination with DFT calculations.
Future plans: Surface stabilization of metal centers with unusual oxidation states and high reactivity for the activation of small molecules. Functionalization of surfaces with reactive tetrapyrrole complexes: On-surface synthesis, electronic interaction, influence of ligands on the electronic structure of surface chemical bond, (electro)catalytic activity.
Figure. (A) Surface reactions of a corrole ligand on a Ag(111) surface. Characterization with (B) XPS and (C) STM. (D) The corrole ligand can be metalated on the surface by reaction with many transition metals (like Fe in this example), resulting in the formation of corrole complexes. (Click to enlarge)
Further reading:
M. Schmid et al., J. Phys. Chem. C 122 (2018) 10392
J.M. Gottfried, Surf. Sci. Rep. 70 (2015) 259
C. Wang et al., Chem. Comm. 50 (2014) 8291
Y. Li et al., J. Am. Chem. Soc. 134 (2012) 6401
W. Hieringer et al., J. Am. Chem. Soc. 133 (2011) 6206
5. Reactivity and Catalytic Properties of Inorganic Nanostructures
This project focuses on the electronic properties, structure and reactivity relationships of metallic and oxidic nanostructures. An example where the catalytic properties of materials depend strongly on the structure and the electronic properties is gold. We therefore investigate a range of model systems with increasing complexity: From single-crystalline systems to nanoporous foams and nanoparticles on oxide supports. The investigations are performed with photoelectron spectroscopy, structure sensitive methods, temperature programmed desorption, and reactivity measurements.
6. Development of Experimental Techniques: Adsorption Calorimeter for the Direct Measurement of Interface Energies
To measure the tiny amounts of heat released during the adsorption of a submonolayer coverages on surfaces of the well-defined bulk materials, sensitive calorimetric techniques combined with pulsed molecular beams are necessary (Figure). We have developed an adsorption calorimeter optimized to measure adsorption energies for large organic molecules and metal complexes. These data represent important benchmark data for the improvement of DFT methods.
Figure. Nanojoule Adsorption Calorimetry. Left: The setup for single-crystalline samples. A thin pyroelectric polymer ribbon is mounted in an arch and pressed gently against the backside of the sample. As a MB pulse of molecules adsorb on the single-crystal sample, heat is deposited and is detected as a temperature change in the pyroelectric polymer. Simultaneously, the sticking probability of the molecular pulse is measured by a mass spectrometer, detecting the reflected fraction of the pulse. Right: The setup for polymer samples, organic films and other polycrystalline samples, which are deposited as thin films directly on the detector. (Figure: J.M. Gottfried, R. Schuster, Surface Microcalorimetry, In: K. Wandelt (Ed.), Surface and Interface Science, Volume 5: Solid-Gas Interfaces I, Wiley-VCH Verlag, 2015.) (Click to enlarge)
7. Development of Experimental Techniques: UHV Ion Beam with RF Mass Filter
In this project, we have developed an apparatus for electrospray ionization ion beam deposition under ultrahigh vacuum conditions. With this technique, it is possible to deposit large organic molecules or biomolecules which are too thermally fragile for sublimation. The collision energy of the ions with the surface can be varied in a wide range. Under hyperthermal conditions (high kinetic energies), reactive collisions occur that can be used for the chemical modification of surfaces. Current projects: Spectroscopic and microscopic investigation of ion beam deposited species; hyperthermal deposition to induce reactive deposition; further surface/interface reactions of these molecules; clarification of mechanistic details of the deposition/neutralization process.
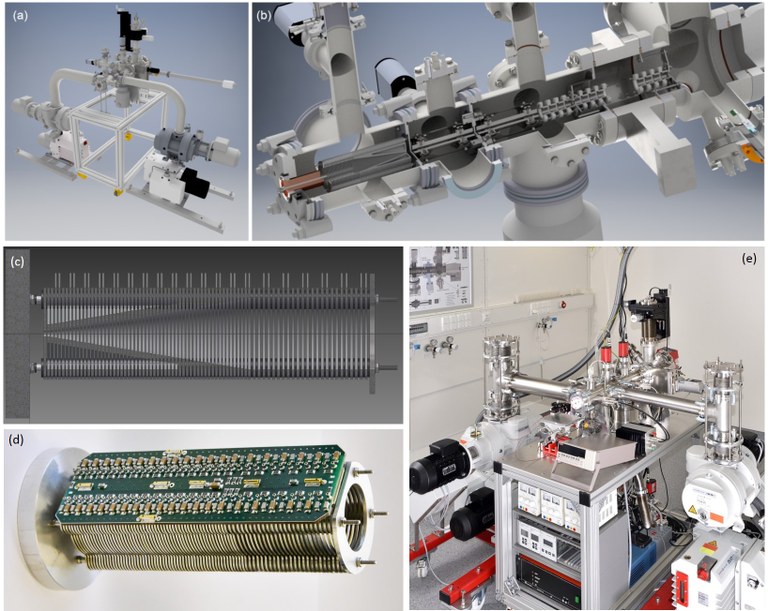